The discovery of the neutron also solved a puzzle that was beginning to vex physicists. When chemists and physicists used their newfound knowledge of protons and electrons to understand the nature of the elements, they were surprised by a strange inconsistency. They discovered that an atom of a given element did not have a fixed weight. For example, in 1932 scientists found that hydrogen came in several flavors. There was ordinary hydrogen—which was thought to be made up of one proton (and one very light electron, whose weight is negligible). Then there was a heavier form of hydrogen that weighed twice as much. They called it deuterium. Soon, they realized there was yet another version that weighed almost exactly three times as much as hydrogen: tritium. All three of these varieties had the same chemistry as hydrogen, but they all had different weights. (And tritium, as it turned out, was radioactive.) Until the neutron was discovered, nothing could explain why a single element could have multiple weights.
Once Chadwick discovered the neutron, though, the answer to the puzzle was obvious. Scientists already knew that the number of protons determined the chemical properties of an atom; hydrogen, deuterium, and tritium each had a single proton in the nucleus, so they were almost identical, chemically speaking. But neutrons can also sit in an atom’s nucleus. Because neutrons don’t have a charge (and don’t attract extra electrons), they don’t affect an atom’s chemical behavior; an extra neutron doesn’t turn hydrogen into a different element. But an extra neutron makes that hydrogen weigh more than before.
Ordinary hydrogen’s nucleus is simply one proton. It weighs as much as one proton, so it is known as hydrogen-1, or
1
H. Deuterium’s nucleus, too, has one proton. But it also has a neutron that weighs roughly the same as the proton; the mass of the nucleus (hence, the mass of the atom) is doubled. Deuterium is thus known as hydrogen-2,
2
H. Tritium has a single proton in its nucleus, but in addition it has two neutrons, making it three times as heavy as ordinary hydrogen. Tritium is therefore designated hydrogen-3,
3
H. All these atoms are considered to be varieties, or
isotopes
, of hydrogen. In a chemical reaction, all three behave more or less the same way. But they have slightly different physical properties by virtue of their nuclei’s different weights.
Scientists were thrilled when they discovered the neutron because it gave them a complete model to explain an atom’s chemical behavior. Just figure out how many protons and neutrons are in a given atom and you can predict its properties extremely well.
Despite the spectacular success of atomic theory, scientists, in some sense, were astonished that atoms could exist at all. Nuclei are finicky things, and it is amazing that any of them are stable. By rights, they should fly apart instantly. They are filled with positively charged protons, and positively charged things repel one another. If the protons in a nucleus were to obey their electrical urges, they would flee each other’s presence, and the nucleus would explode in all different directions. But something forces the protons to stay put and in close proximity to one another. A very strong force—stronger than gravity, stronger than electromagnetism—glues nuclei together, trapping protons inside. In a great burst of creativity, scientists dubbed this strong force . . . the
strong force
. This force holds the secret to nuclear fusion.
The strong force is powerful enough to overcome the natural repulsion that protons have for other protons. However, it can do so only under a fairly narrow range of conditions. If there is the right balance of particles in the nucleus—the correct number of protons and neutrons—the strong force keeps the nucleus stable (or nearly so), preventing the nucleus from exploding. If there are too many neutrons or too few, the nucleus will be unstable. An unstable atom will destroy itself somehow, changing the balance of particles in its nucleus until the nucleus reaches a more stable state. A nucleus can break apart, spit a particle out, or swallow one to get closer to an ideal, stable balance of protons and neutrons.
For example, hydrogen (one proton) and deuterium (one proton and one neutron) are stable. Left to their own devices, they would not change at all. But add a second neutron to the mix, making tritium, and the atom has too many neutrons for comfort. It is no longer stable. Eventually, a tritium atom will, spontaneously, transmute one of its neutrons into a proton (and spit out an electron in the process). The substance left behind is no longer tritium; it has become helium-3, a stable if rare isotope of helium that has two protons and one neutron. (Most helium is helium-4, which has two protons and two neutrons.) It takes an average of twelve years or so for any given tritium atom to undergo this
decay
process, but over time, if you have a jar full of hydrogen-3, you will find that it slowly transforms itself into helium-3.
This transformation process releases energy, because the helium- 3 does not weigh exactly the same as the tritium did. The neutron that disintegrated weighed more than the proton and the electron that it turned into.
17
There is mass missing: it was converted into energy, just as
E
=
mc
2
says. As the unstable tritium changed itself into the stable helium-3, it lost a little bit of mass and released a bunch of energy.
This is an example of a general rule. When a nucleus converts itself from a less-stable variety to a more-stable one, it releases a little bit of energy because some of its mass disappears. And nuclei always “want” to become more stable, just as a ball perched on a hill “wants” to roll down to the bottom. In the process of getting more stable, an atom releases energy, just as a ball rolling down a hill picks up more and more speed as it goes.
Marie Curie was seeing this process with radium. Radium-226 is a heavy atom with 88 protons and 138 neutrons. It is almost stable ... but not quite. On average, after 1,600 years, a radium-226 nucleus spits out an alpha particle (a helium-4 nucleus: two protons and two neutrons), leaving behind 86 protons and 136 neutrons—radon-222—and releasing a bunch of energy. This energy heats the hunk of radium, and it is why Curie observed that chilled radium would warm itself. It is also why a hunk of radium emits radon and helium. It so happens that radon, itself, is unstable; it decays into thorium, releasing energy, which, in turn, decays into another species and another and another, emitting energy at each step. Like a ball rolling down a bumpy hill, it keeps rolling and rolling until it reaches a stable place to rest: in this case, lead-206, which is much more stable than radium-226. The ball has rolled a long way down the hill. But it didn’t roll
all
the way down. There are, in fact, nuclei more stable than lead-206. At the very bottom of the valley are the most stable atoms of them all: the iron group.
Iron-56 (26 protons, 30 neutrons), nickel-62 (28 protons, 34 neutrons), and a few other nearby iron and nickel isotopes are the ne plus ultra of the nucleus world. They are the most stable elements of them all. They are at the very bottom of the valley. All other atoms “want” to be iron, just as a ball anywhere on the slope of a hill “wants” to be at the very bottom.
The landscape of nuclei is very much like a valley with a steep hill on one side and a shallow hill on the other (see the graph on page 47). At the very bottom of the valley is iron. Heavy nuclei, like radium and uranium, have more protons and neutrons than iron—they are high up on the shallow hill. To roll down to iron, they have to get lighter, shedding those extra protons and neutrons. Sometimes they do it in small steps, like radium does. Sometimes they do it violently, by breaking into two or more parts: fission. (Indeed, fission is little more than a way for heavy atoms to roll quickly down the shallow hill, losing mass and releasing energy in the process.) Light elements, on the other hand, are on the steep hill. They have to get bigger if they want to get into the valley of iron. This is what fusion is all about. Two light nuclei, if they slam together, can stick to one another to create a larger nucleus.
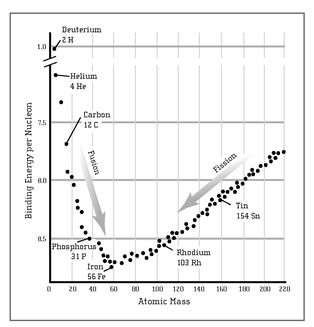
CURVE OF BINDING ENERGY:
Iron is at the bottom of the valley. Light elements on the left and heavy elements on the right release energy when they move down the valley of iron by fusing or fissioning.
Fusion is a way for light atoms to roll down the steep hill toward iron. Since the fusion hill is much steeper than the fission one, a fusion reaction yields much more energy than an equivalent fission reaction. Fusion and fission are two sides of the same coin, but as Teller well knew, fusion is more powerful than fission.
In the 1930s, fusion was soon to solve the puzzle that had so vexed Darwin and Kelvin, and it would answer a question that had bothered humans for millennia: Why does the sun shine? Hans Bethe, then a physicist at Cornell University, would uncover the answer.
In retrospect, William Thomson was fundamentally correct. If the sun were, in fact, an incandescent ball of liquid, the energy released by infalling matter would only power it for a few tens of millions of years, far short of the time that Darwin’s theory needed to explain the diversity of life on Earth (and far short of the time that other scientists needed to explain geological processes). But Thomson’s work preceded
E
=
mc
2
by decades; nobody had yet puzzled over the nature of radioactivity or understood how fission and fusion turn matter into energy. Fusion held the solution to Thomson’s puzzle and vindicated Darwin. Fusion is the source of the energy that has powered the sun for billions of years.
The clues were already old by the time Bethe set to work on the problem. By carefully analyzing the colors of the light that streams from the sun, scientists already had a pretty good idea of what the sun was made of. Roughly 90 percent of its atoms are hydrogen. About 9 percent are helium atoms; in fact, it was by looking at the sun that scientists discovered helium in the first place. The remaining 1 percent is mostly carbon, nitrogen, oxygen, neon, and a tiny smattering of heavier elements, but almost all of these are lighter than iron. The sun bears all the hallmarks of being powered by fusion. Bethe figured out precisely how that power is generated.
A star begins its life as a cloud of gas: mostly hydrogen and a little bit of helium. Because atoms have mass, they attract each other gravitationally, and because of this mutual attraction, the cloud begins to collapse under its own gravity. As gravity compresses the cloud, the cloud heats up.
If gravity were the only force at play, the cloud would simply get smaller and smaller and eventually collapse into a tiny, massive point. But that is not what happens. As the gas cloud gets denser, atoms of hydrogen bump into each other more and more frequently. The collision rate increases dramatically. And as the cloud heats up, its atoms have more energy and collide more violently. The hydrogen atoms jostle each other harder and harder.
Ordinarily, nuclei try to escape from one another. They are positively charged, so they find other nuclei repulsive. When two atoms “collide,” they don’t usually come into physical contact. Once they get within close range, the repulsive forces send them zooming in opposite directions before they actually touch—something like what happens when you try to make two powerful magnets touch each other despite their mutual repulsion. But if the nuclei are moving fast enough—if both atoms are hot enough—then even the mutual repulsion is not enough to keep the nuclei from hitting each other. The two nuclei slam together with great force. This is where fusion begins, and how a sun sparks to life.
Hans Bethe realized that with all these hydrogen nuclei constantly slamming into one another, two hydrogen nuclei—two protons—might smash together at the same time that one spits out a set of particles, turning itself from a proton into a neutron. They fuse, creating deuterium and releasing energy in the process. The deuterium slams into another proton, making helium-3, and again releasing energy. And when two helium-3 atoms collide with each other, they fuse, making helium-4 and releasing two protons and yet more energy. This process, known as the proton-proton chain, turns four hydrogen atoms into helium-4 and lots of energy. Bethe figured out that this was one way our sun generates power: by turning hydrogen into helium. He also realized that other processes are going on as well; for example, the trace amounts of carbon, nitrogen, and oxygen are involved in a cycle that has the same outcome as the proton-proton chain: this process takes four hydrogens and turns them into helium-4. Once a cloud of hydrogen gets hot enough and dense enough, it turns into a machine that converts hydrogen to helium, releasing energy. That is how the nuclear furnace at the heart of a star works.
